Superconductivity is one of the most fascinating and mysterious phenomena in quantum physics. It describes a state in which a material can conduct electricity without resistance, meaning no energy is lost as heat. This remarkable property enables superconductors to power some of the most advanced technologies in medicine, computing, and energy transmission.
Yet, superconductivity comes with a major limitation—it only occurs at extremely low temperatures, often just a few degrees above absolute zero (-273.15°C). This requirement for extreme cooling makes superconductors difficult and expensive to use in everyday applications. Scientists around the world are searching for materials that can achieve superconductivity at room temperature, a discovery that would revolutionize industries from quantum computing to power grids.
A recent breakthrough in this quest comes from a team of physicists at Caltech, who have uncovered a previously unknown superconducting state. Their findings, published in Nature on March 19, provide new insights into the complex mechanisms that enable superconductivity. This discovery could bring scientists one step closer to the ultimate goal of high-temperature superconductors.
How Superconductivity Works
To understand superconductivity, it helps to first look at how normal electrical conductivity functions. In an ordinary metal like copper or silver, electrical current is carried by electrons moving through a lattice of metal atoms. As these electrons travel, they frequently collide with the atoms, losing energy in the process. This energy loss appears as resistance, which is why wires heat up when carrying electricity.
Superconductors, however, behave in a completely different way. When cooled below a critical temperature, the electrons inside them form pairs known as Cooper pairs. These pairs move in a coordinated, wave-like fashion, allowing them to flow through the material without scattering off atoms. Since there is no resistance, an electrical current in a superconducting loop can theoretically flow forever without any energy loss.
The reason this happens lies in quantum mechanics. Electrons in a superconductor experience a weak attraction to each other, creating Cooper pairs. This pairing forms an “energy gap” that prevents electrons from scattering off impurities or lattice vibrations. As long as the electrons remain within this energy gap, they maintain their superconducting state.
The Search for High-Temperature Superconductors
For over a century, scientists have been trying to find materials that can superconduct at higher temperatures. The first discovered superconductors, such as mercury, required cooling to around 4 Kelvin (-269°C) using liquid helium. Later discoveries, including ceramic-based high-temperature superconductors in the 1980s, raised the threshold to around 138 Kelvin (-135°C). These materials can be cooled with liquid nitrogen, which is far cheaper than liquid helium.
However, the holy grail of superconductivity remains room-temperature superconductors—materials that exhibit superconductivity at or near 300 Kelvin (27°C). If such materials could be discovered and manufactured affordably, they would revolutionize energy transmission, allowing power grids to operate with zero loss. They could also enable powerful, highly efficient quantum computers and lead to breakthroughs in medical imaging and transportation technologies, such as maglev trains.
The recent discovery by the Caltech team sheds new light on how superconductivity forms at the microscopic level, potentially bringing researchers closer to understanding how to manipulate it at higher temperatures.
A New Superconducting State: Cooper-Pair Density Modulation
For decades, scientists assumed that a superconductor’s energy gap was uniform across the material. However, in the 1960s, researchers theorized that some superconductors might have a spatially varying energy gap—stronger in some regions and weaker in others. This idea was further developed in the 2000s with the introduction of the pair density wave (PDW) state, a theory suggesting that the superconducting gap could fluctuate over long wavelengths.
Now, the Caltech team, led by Professor Stevan Nadj-Perge, has made a groundbreaking discovery that takes this idea even further. Working with thin flakes of an iron-based superconductor (FeTe₀.₅₅Se₀.₄₅), the researchers observed a new type of superconducting modulation at the smallest possible scale—the atomic level.
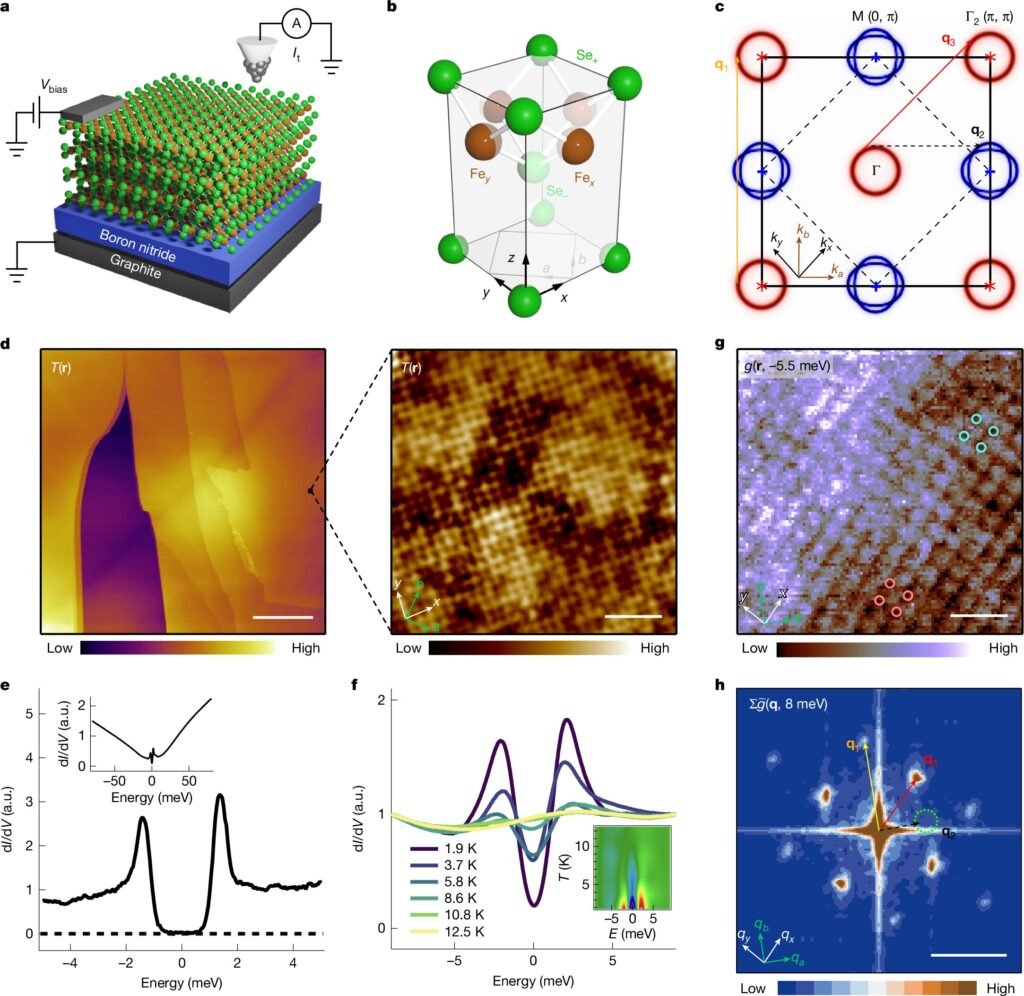
They named this new phenomenon Cooper-pair density modulation (PDM). Unlike previously theorized pair density waves, the PDM state exhibits variations in superconducting strength that match the atomic spacing of the material itself. This means that the superconducting gap oscillates at an atomic scale, with regions of stronger and weaker superconductivity appearing across the lattice.
“The observed gap modulation, reaching up to 40%, represents the strongest reported so far, leading to the clearest experimental evidence to date that gap modulation can exist even at the atomic scale,” says Lingyuan Kong, lead author of the new paper.
A Technological Breakthrough in Imaging Superconductors
One of the biggest challenges in studying superconductors is obtaining a clear, uncontaminated view of their atomic structure. For nearly two decades, researchers struggled to use scanning tunneling microscopy (STM)—a technique that images surfaces at an atomic scale—on iron-based superconductors. The problem was that these materials suffered from severe surface contamination, making it impossible to get clean, reliable data.
The Caltech team, working at the Kavli Nanoscience Institute, developed a new experimental method that allowed them to prepare an ultra-clean surface. This breakthrough finally enabled STM imaging of the material’s superconducting properties, leading to the discovery of the PDM state.
Their work highlights the importance of advanced experimental techniques in pushing the boundaries of scientific knowledge. By improving how we observe superconductors at the atomic level, researchers can better understand the intricate quantum interactions that govern their behavior.
Theoretical Insights: A New Understanding of Superconductivity
To explain their findings, the team collaborated with theoretical physicists Michał Papaj (University of Houston) and Patrick A. Lee (MIT). Their model suggests that the PDM state arises due to a breaking of sublattice symmetry and a unique rotational symmetry present in thin flakes of the material.
This insight provides a deeper understanding of how superconductivity can manifest in different ways at the microscopic level. More importantly, it opens new avenues for exploring superconductors with engineered properties—possibly leading to materials that can function at higher temperatures.
“Understanding the mechanisms leading to the formation of superconductivity and discovering exotic new superconducting phases is not only one of the most stimulating pursuits in the fundamental study of quantum materials but is also driven by this ultimate dream of achieving room-temperature superconductivity,” says Nadj-Perge.
Implications for the Future
The discovery of the Cooper-pair density modulation state is more than just an academic curiosity—it has profound implications for the future of technology.
Quantum Computing: Superconducting qubits, the building blocks of many quantum computers, rely on precisely controlled superconducting states. Understanding how superconductivity can vary at the atomic level may allow scientists to design more stable and efficient qubits.
High-Efficiency Power Grids: If room-temperature superconductors could be realized, power transmission could become nearly 100% efficient, eliminating energy losses and drastically reducing the need for expensive cooling.
Medical Imaging: MRI machines use superconducting magnets to generate powerful magnetic fields. More efficient superconductors could lead to smaller, cheaper, and more accessible MRI machines for hospitals worldwide.
New Electronic Devices: The ability to modulate superconductivity at an atomic level could lead to novel electronic components, potentially enabling new forms of ultra-fast, low-power computing.
Conclusion: A Step Closer to Room-Temperature Superconductors?
While room-temperature superconductivity remains an elusive goal, discoveries like the Cooper-pair density modulation state bring us closer to understanding how superconductivity works at its most fundamental level. The work of Nadj-Perge and his team at Caltech represents a significant leap forward, providing experimental proof of a new superconducting phase that challenges long-held assumptions about how Cooper pairs behave.
As research continues, each new discovery adds another piece to the puzzle. With advancing technology and deeper theoretical understanding, the dream of room-temperature superconductivity may not be as far away as it once seemed.
Reference: Lingyuan Kong et al, Cooper-pair density modulation state in an iron-based superconductor, Nature (2025). DOI: 10.1038/s41586-025-08703-x