Imagine a region of space where the laws of physics teeter on the edge of breakdown—where atoms collapse, matter is crushed beyond recognition, and magnetic fields reach intensities no human-made experiment could ever hope to match. Now, picture two of these extreme objects—neutron stars—spiraling towards each other in a cosmic death dance. As they collide, they unleash a storm of gravitational waves, energetic ripples through the very fabric of spacetime.
These neutron star mergers are among the most awe-inspiring phenomena in the universe, both visually and scientifically. They offer a rare glimpse into the behavior of matter under conditions so extreme that they defy our conventional understanding. And yet, just as scientists begin to decode the secrets locked within these gravitational wave signals, a new variable threatens to complicate the picture—magnetic fields.
What Are Neutron Star Mergers, and Why Do They Matter?
To grasp the significance of these collisions, we must first understand what neutron stars are. Born from the explosive deaths of massive stars, neutron stars are the collapsed cores left behind after a supernova. Composed almost entirely of neutrons packed into an incomprehensibly dense ball—imagine the mass of the Sun squeezed into a city-sized sphere—these objects are the densest form of matter known, second only to black holes.
When two neutron stars orbit one another in a binary system, they slowly spiral inward due to the emission of gravitational radiation, eventually merging in a spectacular and violent event. These mergers are not only cataclysmic; they’re also rich with information. The gravitational waves they emit carry encoded signatures of the neutron stars’ internal structure, composition, mass, and even their thermodynamic properties. Studying these waves can teach us how matter behaves at densities far beyond those achievable in terrestrial laboratories.
This is where the equation of state (EoS) comes in—a theoretical framework describing the relationship between pressure, temperature, and density inside neutron stars. Scientists have long hoped that, by analyzing the gravitational wave spectrum produced in these mergers, they could infer the precise form of the EoS and uncover the true nature of ultradense matter.
The Hidden Complexity: Magnetic Fields Step Into the Spotlight
Until recently, most theoretical models and simulations seeking to decode neutron star mergers focused primarily on the EoS, often treating magnetic fields as a secondary concern. But in a groundbreaking study led by researchers from the University of Illinois Urbana-Champaign and the University of Valencia, this assumption is now being challenged.
Their simulations—published in the prestigious Physical Review Letters—reveal that magnetic fields aren’t just background noise. In fact, these magnetic fields can drastically alter the frequencies of the gravitational waves produced after the merger, thus complicating any attempt to interpret the data purely through the lens of the EoS.
“The frequencies encode many of the characteristics of the neutron stars,” explained Antonios Tsokaros, lead author of the study. “Identifying them correctly will enable us to understand many of the yet unknown properties of these extraordinary objects.”
But what if magnetic fields change those frequencies in subtle, yet significant ways?
A Spoonful of Neutron Star: The Weight of the Unknown
One of the more mind-bending aspects of neutron stars is their density. A mere teaspoon of neutron star matter weighs as much as Mount Everest. The internal composition of such an object—dominated by neutrons, and perhaps even exotic phases of matter like hyperons or quark-gluon plasma—remains a deep mystery. The EoS provides a mathematical bridge into this hidden world, offering predictions about how matter behaves when squeezed to such extremes.
By analyzing how gravitational waves change depending on different assumed EoSs, researchers can reverse-engineer the properties of the star’s core. But if magnetic fields are strong enough to affect these same wave frequencies, the interpretation becomes murky. Are frequency shifts due to the EoS, or the magnetic field?
That’s the question Tsokaros and his team set out to answer.
Simulating Stellar Mayhem: The Role of Magnetic Topology
To explore the interplay between magnetic fields and oscillating frequencies, the research team performed detailed general relativistic magnetohydrodynamic (GRMHD) simulations. These are cutting-edge computer models that incorporate Einstein’s theory of general relativity, fluid dynamics, electromagnetism, and more.
The researchers experimented with various combinations: two different equations of state, different neutron star masses, and three distinct magnetic field configurations or “topologies.” These topologies represent how the magnetic fields are distributed and evolve during the merger process. The results were striking.
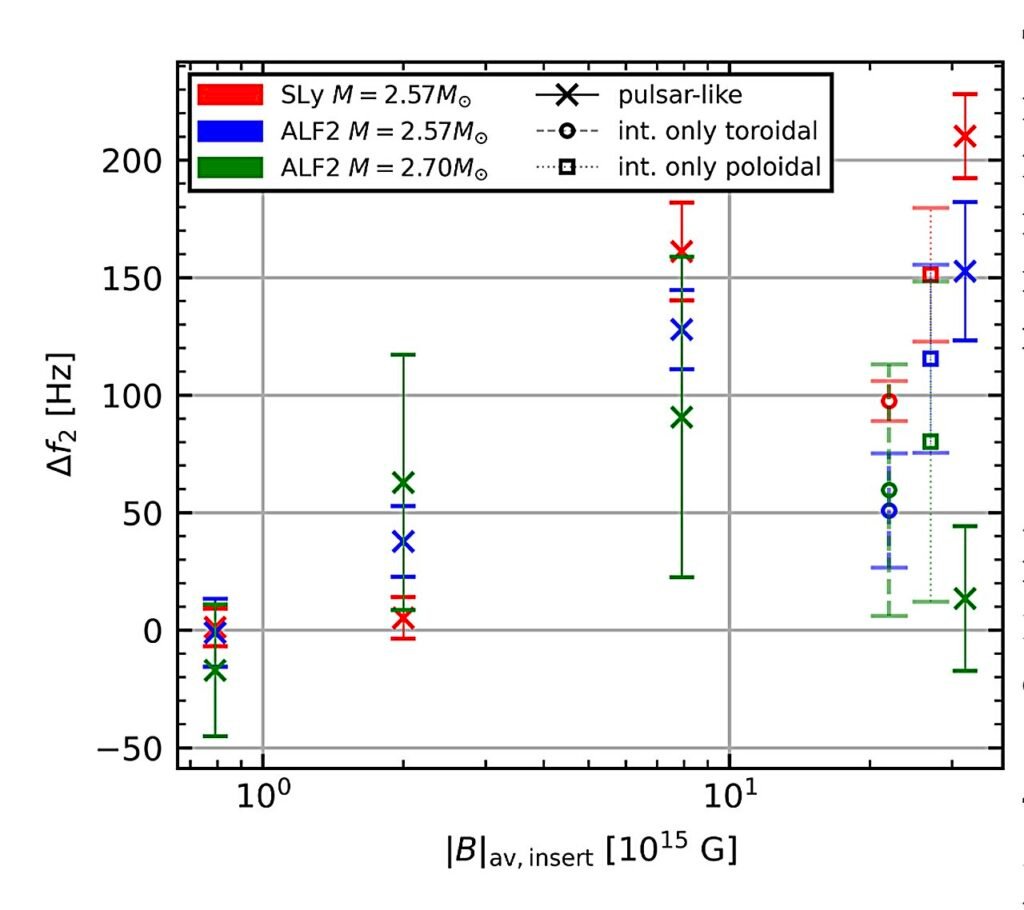
According to Jamie Bamber, a postdoctoral researcher involved in the study, the simulations showed that during the merger, the magnetic field is not just sustained—it is amplified to titanic levels, more than a billion times stronger than anything humans have ever produced.
These extreme magnetic fields trigger their own set of oscillations within the post-merger object—oscillations that produce gravitational waves at higher frequencies than expected. In short, the magnetic field masks or distorts the frequency signatures that physicists typically associate with the equation of state.
“This makes the interpretation of observations more complicated than previously thought,” Bamber noted. “The magnetic field causes the merger remnant to oscillate and produce gravitational waves at a higher frequency.”
Why the Magnetic Field Matters for the Next Generation of Observatories
This revelation comes at a critical time. Since the first detection of gravitational waves from a neutron star merger in 2017 by LIGO, scientists have entered a new era of multi-messenger astronomy—observing cosmic events not just through light, but through gravitational waves and particles. That 2017 event, GW170817, was observed simultaneously with gamma-ray bursts by NASA satellites, providing a treasure trove of data.
Yet even that data is just the tip of the iceberg. Future observatories, such as the Einstein Telescope and the Cosmic Explorer, will be significantly more sensitive and capable of detecting the high-frequency oscillations that arise immediately after a neutron star merger.
As Tsokaros emphasizes, “Next-generation gravitational wave observatories… will be able to detect the actual merger of two neutron stars as they form a single rotating compact object and the various frequencies of oscillations associated with the merger process.”
But those observations will only be meaningful if scientists can correctly interpret what they’re hearing. Think of it like trying to understand a symphony where multiple instruments are playing at once—if you don’t know which sounds come from which instruments, the entire piece can be misread.
A New Layer of Complexity, a New Horizon of Discovery
Professor Milton Ruiz, another co-author of the study, underscores the stakes: “To make an accurate assessment of the post-merger phase… one needs to include the effects of the magnetic field. Failing to do so may lead to erroneous conclusions about the physical properties of the system.”
This means that any model hoping to decode gravitational wave signals from neutron star mergers must account for magnetic fields from the outset. It’s not enough to study the EoS in isolation anymore—the two are inextricably linked.
Moving forward, Tsokaros and his team plan to extend their simulations to even higher resolutions than previously possible, thanks to advances in computational power and algorithms. These enhanced models will provide even finer details about how different magnetic field strengths and geometries influence gravitational wave signatures.
Beyond the Horizon: The Future of Neutron Star Astrophysics
The implications of this research are profound. Neutron star mergers aren’t just exotic events; they are natural laboratories that may help solve some of the greatest mysteries of modern physics. From understanding the origin of heavy elements like gold and platinum, to probing the limits of nuclear matter, to potentially uncovering new phases of matter, these mergers are a goldmine of knowledge.
And yet, as this latest research shows, nature doesn’t give up its secrets easily. Each discovery peels back one layer of mystery while revealing several more. The inclusion of magnetic fields adds another dimension—literally and figuratively—to our understanding of these cosmic collisions.
As Professor Stuart Shapiro puts it, “Many of the signature features of these simulations will only be identified by the next generation of gravitational wave detectors… which will detect the high frequencies associated with the merger and post-merger of binary neutron stars.”
Conclusion: Listening to the Universe’s Deepest Secrets
In the end, the study of neutron star mergers is more than just a scientific endeavor. It’s a journey to understand the very limits of reality—a place where time warps, matter behaves like liquid metal under crushing gravity, and light struggles to escape. The gravitational waves that echo from these events are like notes in a universal song—notes shaped by density, heat, spin, and now, as we’ve come to realize, powerful magnetic fields.
To truly decipher that cosmic symphony, we must be willing to listen more carefully, to account for every instrument, every variable, no matter how complex. Only then can we hope to answer the deepest questions about the universe: What is matter? What are we made of? And how does the cosmos, in its wildest moments, truly behave?
Reference: Antonios Tsokaros et al, Masking the Equation-of-State Effects in Binary Neutron Star Mergers, Physical Review Letters (2025). DOI: 10.1103/PhysRevLett.134.121401. On arXiv: DOI: 10.48550/arxiv.2411.00939