In a stunning leap for astrophysics and laboratory plasma physics, researchers at the University of Science and Technology of China (USTC) have accomplished what has long evaded direct observation: they have witnessed, in real-time and under controlled laboratory conditions, the acceleration of ions through reflection off magnetized collisionless shocks—a cosmic mechanism suspected to lie at the heart of some of the most energetic phenomena in the universe. This momentous study, recently published in Science Advances, brings us closer than ever to understanding how the universe supercharges particles to nearly light speeds, and does so with the kind of empirical clarity that scientists have dreamed of for decades.
The Mystique of Cosmic Shocks
Imagine vast clouds of interstellar plasma, supernova explosions blasting waves through space, or solar wind crashing into Earth’s magnetic field—these events are more than just visual spectacles. They’re also natural particle accelerators, hurling protons and ions to energies far beyond the reach of our most powerful machines. At the heart of these cosmic particle accelerators are collisionless shocks—intense, invisible shock fronts that, unlike sound waves in air, do not rely on particle-to-particle collisions but on electromagnetic interactions in plasma.
These shocks have been suspected for years as the prime engine behind the acceleration of cosmic rays, the high-energy particles that bombard Earth from all directions. But one enduring mystery has haunted astrophysicists: How exactly do these particles get their first “kick”? What pushes them hard enough to start their long journey across the galaxy?
The Chicken-and-Egg Puzzle of Cosmic Rays
At the center of this enigma is the Fermi acceleration mechanism, a well-established theory in which particles bounce back and forth across a shock front, gaining a bit of energy with each crossing—like a cosmic pinball machine. Over time, they can build up to tremendous energies. However, Fermi acceleration presumes the particle already has a significant velocity—enough to penetrate the shock barrier. That raises a paradoxical question: What mechanism gives the particle its first burst of energy so it can even begin this process?
This critical question—how particles “get in the door” of the Fermi acceleration process—has driven debate among theorists for decades. Two major contenders have emerged: Shock Drift Acceleration (SDA) and Shock Surfing Acceleration (SSA). Both mechanisms describe how particles might extract energy from the electromagnetic fields around shocks, but they differ fundamentally in how the particle interacts with the shock front. Until now, the field lacked the clarity to declare a winner—spacecraft data are too distant and sparse, and laboratory experiments have lacked the right conditions.
That is, until now.
Recreating a Star’s Fury in a Lab
At the center of this achievement is Shenguang-II, a powerful laser facility in China that allows scientists to mimic astrophysical conditions on a miniature scale. Using this setup, the USTC team orchestrated an experiment worthy of a science fiction epic: they generated a shock wave in a controlled plasma medium that behaved just like the ones found in space.
The researchers launched a “piston” plasma at speeds greater than 400 kilometers per second into a magnetized ambient plasma. This setup produced a quasi-perpendicular, supercritical collisionless shock—the exact kind found at the edge of Earth’s magnetic field and around supernova remnants. It was a tiny replica of a colossal cosmic event, and it was the perfect laboratory for testing long-held theories.
But this wasn’t just a spectacular display of scientific fireworks. The key to the experiment’s power was its diagnostic precision. Using high-resolution tools like optical interferometry and ion time-of-flight detectors, the team captured a detailed snapshot of what was happening at the shock front.
And what they saw was remarkable.
A River of Ions, Racing Against the Shock
Out of the chaos of the plasma clash emerged a quasi-monoenergetic ion beam—a focused stream of ions shooting upstream, away from the shock, at velocities between 1,100 and 1,800 km/s. This was no random spray of particles. These ions had clearly gained energy, and fast. What’s more, their speed was two to four times that of the shock itself.
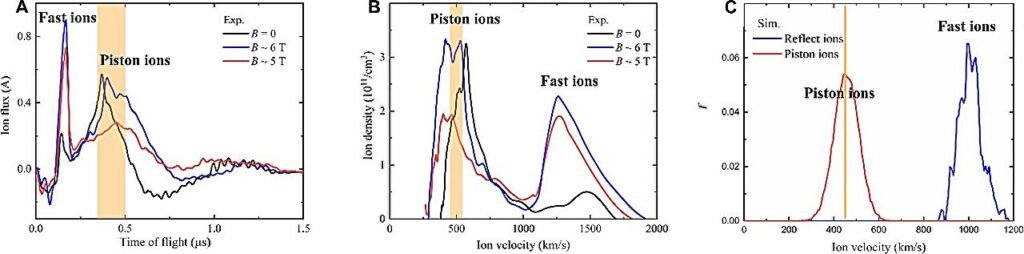
This phenomenon, rarely seen with such clarity, bore a striking resemblance to data collected from Earth’s bow shock, where the solar wind meets the planet’s magnetic defenses. But in this laboratory setting, the data were even cleaner, more precise, and free from the noise of cosmic distance.
Simulating the Invisible: The Role of Particle-In-Cell Modeling
To unravel what exactly happened to those ions, the researchers turned to particle-in-cell (PIC) simulations, a computational technique that models how particles and electromagnetic fields evolve together. These simulations revealed something extraordinary: the ions weren’t just drifting—they were bouncing.
When the fast-moving ions encountered the compressed magnetic field and electrostatic potential of the shock front, they were reflected back upstream. But this wasn’t a simple ricochet. As they reflected, they interacted with the shock’s motional electric field, a crucial feature of SDA. This field, created by the motion of the magnetic shock through the plasma, acts like a conveyor belt, injecting energy into the ions as they move along and across the shock front.
This dynamic interplay—the acceleration along and perpendicular to the shock—produced a high-speed ion beam with a very specific energy signature. It matched precisely what the simulations predicted for shock drift acceleration.
Shock Drift Acceleration Triumphs
This finding is monumental because it decisively rules out shock surfing acceleration as the dominant mechanism—at least under these conditions. SSA relies on ions being caught and accelerated within electrostatic waves at the shock front. But in this experiment, no such waves played a major role in the energy gain. The energy was extracted from the motional electric field, a classic signature of SDA.
In other words, this experiment doesn’t just support the theory of SDA—it confirms it in a real-world scenario. It settles a debate that has lasted for decades, not with speculation, but with hard evidence.
Bridging the Gap Between Earth and the Stars
Perhaps the most impressive aspect of this study is how closely it mirrors actual space environments. The magnetic field strength, clocking in at 5–6 Tesla, and the plasma densities and flow speeds used in the experiment perfectly match the range seen in Earth’s bow shock and in other astrophysical sites. This makes the laboratory results not only relevant but directly comparable to spacecraft measurements.
The ability to create astrophysically accurate plasma conditions in the lab is no small feat. It transforms the way we study space physics. Instead of waiting for rare events or distant probes to gather elusive data, scientists can now recreate—and control—similar conditions right here on Earth. The possibilities are staggering.
Implications for Particle Physics and Fusion
Beyond the theoretical elegance, this experiment has powerful practical implications. For instance, understanding how to inject ions into high-energy beams has direct relevance to laser-driven ion accelerators. These accelerators are used in cancer treatment, materials science, and fundamental physics. With controlled magnetic shocks, scientists might soon be able to fine-tune ion beams for better precision and energy efficiency.
Moreover, the results may help solve some of the persistent problems in inertial confinement fusion (ICF)—a technique that seeks to harness nuclear fusion by compressing fuel pellets with powerful lasers. One of the challenges in ICF is the presence of shock-induced instabilities that disturb the fuel compression. Knowing how shocks behave and how they transfer energy could help mitigate these effects, pushing us a step closer to clean, limitless fusion power.
A New Era for Cosmic Particle Physics
With this experiment, the team at USTC has opened a door—not just to a better understanding of our universe, but to a whole new methodology for exploring it. Their reproducible, tunable shock platform is not just a one-off success. It’s a tool—an experimental sandbox—where fundamental theories of plasma and particle physics can now be tested with precision.
Future experiments could use this platform to explore more complex scenarios: shocks in multi-species plasmas, shocks under turbulent conditions, or even repeated particle-shock interactions that could simulate the full Fermi acceleration cycle. These studies could reveal how cosmic rays achieve their near-light-speed velocities and why certain astrophysical environments are such efficient particle accelerators.
Conclusion: From Laser Labs to the Edge of the Universe
What began as an abstract theoretical question has now become an observable fact. Thanks to lasers, magnetic fields, and brilliant experimentation, scientists have witnessed the birth of accelerated particles in a way that mirrors the grand mechanics of the universe itself.
This isn’t just a technical milestone—it’s a philosophical one. It reaffirms the power of human curiosity and ingenuity. By creating a miniature cosmos in the laboratory, we are not merely modeling the stars—we are learning to speak their language.
As research continues, and as laser facilities grow even more powerful and sophisticated, we can expect the boundary between laboratory and cosmos to shrink further. The stars may be billions of miles away, but thanks to breakthroughs like this, the forces that shape them are now within our reach.
Reference: Hui-bo Tang et al, Laboratory observation of ion drift acceleration via reflection off laser-produced magnetized collisionless shocks, Science Advances (2025). DOI: 10.1126/sciadv.adn3320
Behind every word on this website is a team pouring heart and soul into bringing you real, unbiased science—without the backing of big corporations, without financial support.
When you share, you’re doing more than spreading knowledge.
You’re standing for truth in a world full of noise. You’re empowering discovery. You’re lifting up independent voices that refuse to be silenced.
If this story touched you, don’t keep it to yourself.
Share it. Because the truth matters. Because progress matters. Because together, we can make a difference.
Your share is more than just a click—it’s a way to help us keep going.